Body water accounts for approximately 60 % of body weight; it is mainly distributed in the ECS and ICS. The ICS contains nearly 55 % of total body water and the ECS approximately 45 % (about 15 L in a normal adult). Among the three compartments, the IVS accounts for about 15 % of ECS water, the ISS for nearly 45 %, and the TCS for about 40 % (Agrò and Vennari 2013a) (Fig. 13.2).
The TCS is a functional compartment represented by the amount of fluid and electrolytes continually exchanged (in and out) by cells with the ISS and by the IVS with the ISS (Agrò and Vennari 2013a). Other fluids composing the ECS are secretions, ocular fluid, and cerebrospinal fluid (Agrò and Vennari 2013a; Chappell et al. 2008).
Fluid and electrolyte balance is both an external balance between the body and its environment and an internal balance between the ECS and ICS and between the IVS and ISS. This balance is based on specific chemical and physical properties of body fluids, such as ionic composition, pH, protein content, osmotic pressure, osmolarity, and colloid osmotic pressure (Agrò and Vennari 2013a) (Table 13.1).
Table 13.1
Main properties of body fluids
Properties | Plasma | Interstitial fluid | Intracellular fluid |
---|---|---|---|
Colloid osmotic pressure (mmHg) | 25 | 4 | – |
Osmolality (mOsmol/kg) | 280 | 280 | 280 |
pH | 7.4 | 7.4 | 7.2 |
Na+ (mEqlL) | 142 | 143 | 10 |
K+ (mEqlL) | 4 | 4 | 155 |
Cl− (mEqlL) | 103 | 115 | 8 |
Ca2+ (mEqlL) | 2.5 | 1.3 | <0.001 |
13.1.2 Ionic Balance
Ionic balance is based on the principle of the “electric neutrality”: the sum of cations must be the same of the sum of anions. In other words, the net sum of the electric charge in the body fluids is zero. Ionic composition of ICS and ECS are different and further differences exist in the ECS between the IVS and the ISS (Table 13.1).
In clinical practice, the only value directly measurable is the plasma concentration of each ion is the only value directly measurable. Generally, this value is considered as a reference to evaluate the presence of electrolyte alterations. The relationship between the ionic plasmatic composition and the neutrality principle is expressed by the Gamble gram (Fig. 13.3). Examining the Gamble gram is immediately evident that the sum of cations (Na+ + K+ + Ca++ + Mg++ + others) is 154 mEq/L and is the same of anions (Cl− + bicarbonate + proteins + phosphates + sulfates + organic acids). Na+ and K+ represent 94 % of all IVS cations, while Cl− and bicarbonate represent 84 % of all anions. Na+, K+, Ca++, and Mg++ are generally measured through laboratory exams, while bicarbonate is only calculated by using the Henderson–Hasselbalch equation, when arterial blood sample is performed (Gamble 1947) (see Chap. 15).


Fig. 13.3
Gamble gram. Electric neutrality principle: the sum of plasmatic cations is equivalent to the sum of plasmatic anions
13.1.2.1 Sodium
Sodium is the most highly represented cation in the ECS, and it has a key hemodynamic role: it is the main determinant of ECS volume, contributes to renin–angiotensin–aldosterone system activation, and regulates ADH secretion. Sodium concentration determines body fluid osmolarity. Changes in sodium plasma level are responsible for modification in fluid movement across the body space, determining ICS and ECS volume variation. The normal sodium concentration in plasma and the ISS is about 142 mEq/L, and it is higher than the ICS concentration (10 mEq/L) (Agrò and Vennari 2013a; Miller 2009).
13.1.2.2 Potassium
Potassium is the main cation of the ICS. It plays a central role in determining the resting cell membrane potential, especially for excitable cells such as myocytes. Therefore, it influences the transmission of impulses along the cardiac pacemakers (potentially predisposing to arrhythmias) and the contraction of myocardial cells. It is also involved in a variety of metabolic processes, including energy production and the synthesis of nucleic acids and proteins. The normal potassium concentration in plasma is about 4.5 mEq/L (Agrò and Vennari 2013a; Miller 2009).
13.1.2.3 Calcium
Several extra- and intracellular activities are regulated by calcium action. Calcium is involved in endocrine, exocrine, and neurocrine secretion; coagulation activation; muscle contraction (it has a great inotropic effect); potential membrane depolarization; cell growth; enzymatic regulation; and in the metabolism of other electrolytes (especially potassium and magnesium). The normal calcium plasma concentration is 2–2.6 mEq/L. Calcium may circulate in the plasma bound to albumin and free from proteins. Free calcium may be ionized (physiologically active) or nonionized (chelated with inorganic anions such as sulfate, citrate, and phosphate). The amounts of the three forms are altered by many factors, such as pH, plasma protein levels (hypoalbuminemia reduces total calcium, but not free fraction), and percentage of anions associated with ionized calcium (blood products contain citrate) (Agrò and Vennari 2013a; Miller 2009).
13.1.2.4 Magnesium
Magnesium is the physiological antagonist of calcium. It plays a crucial role in neuromuscular stimulation and modulation of excitable cells activity (membrane stabilizing activity); it also acts as a cofactor of several enzymes involved in the metabolism of three major categories of nutrients: carbohydrates, lipids, and proteins. The normal plasma concentration is about 0.85–1.25 mEq/L (Agrò and Vennari 2013a; Miller 2009).
13.1.2.5 Chloride
Chloride is the most important anion of the ECS. Together with sodium, it determines the ECS volume. It is also responsible for the resting potential of the membrane and action potential, acid–base balance, and plasma osmotic pressure. The normal plasma chloride concentration is 97–107 mEq/L (Agrò and Vennari 2013a; Miller 2009).
13.1.2.6 Bicarbonate
Bicarbonate is the main buffer system of the blood. It plays a crucial role in maintaining acid–base balance. Two-thirds of the CO2 in the human body is metabolized as bicarbonate, through the action of carbonic anhydrase. The equilibrium between CO2 and bicarbonate leads to the elimination of volatile acid. The bicarbonate buffer system is described by the following equilibrium reaction (Agrò and Vennari 2013a):


When there is an increased concentration of H+, the system reacts by shifting the reaction equilibrium to the left (towards the production of CO2), while when the concentration of H+ is reduced, the system moves to the right, resulting in the production of H+. The bicarbonate buffer system works “in concert” with several organs (see Chap. 15). Bicarbonate has a normal plasma concentration of about 24 mmol/L (Agrò and Vennari 2013a).
13.1.3 Osmolar Balance
Chemical properties of ICS and ECS are very different (Table 13.1). According to their Na+ and glucose concentration, ICS is sweet, while ECS is salty. Despite such difference, the principle of iso-osmolarity is crucial for body balance: ICS osmolarity and ECS osmolarity must be the same. Osmotic pressure (μ) is the force exerted by the sum of osmotically active particles (Na+ and other electrolytes) that do not freely pass through semipermeable membranes (Agrò and Vennari 2013a). According to osmotic pressure difference among the compartments, water freely passes form ICS to ECS, and vice versa, in order to achieve the equilibrium (Agrò and Vennari 2013a) (Fig. 13.4). In particular, water shifts from the body compartment with lower osmotic pressure to that with higher osmotic pressure. The osmotic pressure gradient between the two compartments is described as tonicity (Agrò and Vennari 2013a; Voet et al. 2001).


Fig. 13.4
Osmotic pressure. According to osmotic pressure, water diffusion will take place from low to high electrolytic concentration (From Agrò and Vennari 2013a, pp. 1–26)
The osmotic pressure of the plasma osmotic pressure is 288 ± 5 mOsm/L, and it can be calculated by measuring the plasma concentrations of Na, glucose, and urea.
![$$ \begin{array}{c} Plasma\; osmotic\; pressure=2\times \left[N{a}^{+}\right]\;\Big( mmol/L\Big)+ urea/2,8\;\Big( mg/ dL\Big)\\ {}+ glucose/18\;\left( mg/ dL\right)\end{array} $$](/wp-content/uploads/2017/02/A305677_1_En_13_Chapter_Equb.gif)
![$$ \begin{array}{c} Plasma\; osmotic\; pressure=2\times \left[N{a}^{+}\right]\;\Big( mmol/L\Big)+ urea/2,8\;\Big( mg/ dL\Big)\\ {}+ glucose/18\;\left( mg/ dL\right)\end{array} $$](/wp-content/uploads/2017/02/A305677_1_En_13_Chapter_Equb.gif)
The difference between measured osmolarity and calculated osmolarity is called the osmolar gap. A high osmolar gap suggests either the presence of an exogenous compound (e.g., ethanol) whose identity should be sought or the elevation of endogenous constituents that may not have been measured (e.g., proteins, ketoacids, lipids) (Hendry 1961).
The main determinant of plasma osmolarity is Na+. Sodium salts are responsible for the 95 % of the whole plasma osmotic pressure. Since osmotic pressure determines water tendency to move in or out of the cell, the Na+ concentration is the main determinant of the relative volumes and hydration of the ICS and ECS (Voet et al. 2001). When the ECS osmotic pressure increases (i.e., hypernatremia), water immediately passes from ICS to ECS to restore osmotic equilibrium, leading to a reduction in ICS volume and an increase in ECS volume, while the opposite movement develops when ECS osmotic pressure is reduced (i.e., hyponatremia), with an increase in ICS volume and a reduction in ECS volume (Agrò and Vennari 2013a). Sodium and water movements are further related by the mechanism of regulation of osmolarity and volemia. They are hormonal systems such as ADH, renin–angiotensin–aldosterone (RAA) system, ANP, BNP, and the thirst. Although with different thresholds of sensibility (i.e., ADH is more sensible to osmolarity, while RAA system to volemia), they are all enrolled both in case of osmolarity and volemia alterations (Agrò and Vennari 2013a). Being the sole organ able to divide water movement from Na+ movement, the kidney is the main target of the regulatory systems. When an increase in plasmatic osmotic pressure develops, ADH and the thirst promote water reabsorption and intake, while when plasmatic osmotic pressure reduces, the reduction in water intake and its increased kidney excretion restore normo-osmolarity (Agrò and Vennari 2013a) (Fig. 13.5).


Fig. 13.5
Mechanism of ADH secretion: when fluid volume decreases plasma sodium concentration and plasmatic osmolarity increases, leading to hypothalamic osmoreceptor stimulation. The hypothalamus will then stimulate the posterior pituitary gland that releases antidiuretic hormone. ADH will make renal distal tubules able to reabsorb water into the IVS in order to maintain homeostasis of fluid balance. ADH secretion is more sensible to plasmatic osmolarity than circulating blood (From Agrò and Vennari 2013b, pp. 71–92)
The RAA system is manly enrolled in sodium and water renal reabsorption, when hypovolemia develops. In case of hypervolemia, the ANP and the RAA system inhibition lead to excretion of sodium and water overload (Agrò and Vennari 2013a) (Fig. 13.6).


Fig. 13.6
Renin–angiotensin–aldosterone system: hypovolemia reduces perfusion of juxtaglomerular apparatus, with renin release. Circulating renin converts angiotensinogen to angiotensin I; subsequently ACE (angiotensin-converting enzyme) acts on angiotensin I converting it to angiotensin II. This hormone increases NSS activity, increases reabsorption of Na and water by kidneys directly and through aldosterone action, and determines vasoconstriction and ADH secretion (From Agrò and Vennari 2013b, pp. 71–92)
13.1.4 Fluid Movement Through Capillary Membranes
Fluid movements across capillary membrane are regulated by physical forces and specific properties of the semipermeable membranes (Agrò and Vennari 2013a).
Accordingly, electrolyte and protein concentrations, as well as osmotic properties, play a crucial role, as expressed in the Starling equation:
![$$ Jv={K}_f\;\left([{P}_c-{P}_i)\hbox{--} \sigma\;\left[{\delta}_c-{\delta}_i\right]\right) $$](/wp-content/uploads/2017/02/A305677_1_En_13_Chapter_Equc.gif)
![$$ Jv={K}_f\;\left([{P}_c-{P}_i)\hbox{--} \sigma\;\left[{\delta}_c-{\delta}_i\right]\right) $$](/wp-content/uploads/2017/02/A305677_1_En_13_Chapter_Equc.gif)
According to this equation, water flow depends on six variables:
1.
Capillary hydrostatic pressure (P c)
2.
Interstitial hydrostatic pressure (P i)
3.
Capillary oncotic pressure (δ c)
4.
Interstitial oncotic pressure (δ i)
5.
Filtration coefficient (K f)
6.
Diffusion coefficient (σ)
The equation states that the net filtration (Jv) is proportional to the net driving force ([P c − P i] − σ [δ c − δ i]). If this value is positive, water leaves the IVS (filtration). If it is negative, water enters or remains in the IVS (absorption) (Fig. 13.7). A modification of only one of the forces involved in the Starling equation may alter fluid movement across body compartment (Agrò and Vennari 2013a).


Fig. 13.7
Starling forces. On the arterial side of capillary vessels, forces putting out water overcome those putting in. On the venous side is the contrary (Modified from Agrò and Vennari2013a, pp. 1–26)
13.2 Basis of Fluid and Electrolyte Pathophysiology in the Postoperative ICU Setting of Cardiac Surgery
13.2.1 Alterations in Water Distribution
Adequate fluid replacement in cardiac patients is fundamental to the successful of surgery. In patients undergoing cardiac surgery, a correct fluid management maintains an adequate circulatory volume and a proper electrolyte and acid–base balance, avoiding arrhythmic (i.e., atrial fibrillation), hemodynamic (i.e., hypotension, pulmonary edema), and other complications (Agrò et al. 2013a).
Hypovolemia is a frequent occurrence among cardiac surgical patients. Fluid imbalance may be due to an absolute volume deficit or to a relative volume deficit and may be associated to concurrent electrolyte and acid–base problems (Agrò et al. 2013a).
Absolute volume deficits can be due to hemorrhage (i.e., coagulation and platelet impairment, surgical complications) or to severe dehydration issues (i.e., diuresis induced by mannitol often used during cardiopulmonary bypass), while relative volume deficits can be due to vasodilatation caused by sedation, systemic inflammatory response syndrome (SIRS) due to surgical stress and cardiopulmonary bypass (CPB), and by rewarming (Tommasino et al. 1988). In this case, there is a shift of fluid from IVS to ISS. Hypovolemia develops in absence of obvious fluid loss, leading to a reduction in cardiac index (CI), tissue perfusion, and O2 delivery (DO2) with a high risk of organ failure, potentially fatal (Agrò et al. 2013a).
Another cause of concern is the risk of fluid overload, which may precipitate or worsen the cardiac function (especially in patients with impaired ventricular contractility or compliance), leading to acute pulmonary edema and/or cardiogenic shock. These events may complicate patient management, requiring the use of inotropes and other cardiovascular active drugs. Moreover, a fluid overload may lead to interstitial edema, compression of microvasculature, and an increased oxygen diffusion distance, compromising DO2 and O2 diffusion to tissues (Fanzca 2012). Finally, aggressive perioperative fluid administration may cause hemodilution, with an increased need for blood products (Vretzakis et al. 2011).
In order to avoid both hypovolemia and fluid overload, stabilization of the cardiovascular system through a rational fluid therapy should take into account the type of surgery and the mechanism of fluid movement among body compartments (Stephens and Mythen 2003; Adams 2007).
According to Starling equation, ISS volume is generally maintained by lymphatic drainage. Any flux of fluid into the ISS increases its hydrostatic pressure and decreases its oncotic pressure, limiting the development of interstitial edema. In literature many works demonstrated that the Starling equation does not fully explain the movement between IVS and ISS across the endothelial membrane (Fanzca 2012; Adams 2007; Hu and Weinbaum 1999; Hu et al. 2000; Levick 2004). The deviation from theory is due to a meshwork of membrane bound, negatively charged glycoproteins and proteoglycans, called endothelial glycocalyx.
The endothelial glycocalyx is a dynamic structure continuously degraded and resynthesized. It is composed by proteins produced by endothelial cells or entrapped from the plasma. Glycocalyx is a barrier for larger molecules and probably is mainly responsible for the oncotic gradient across IVS and ISS (Fanzca 2012). Moreover, it prevents the endothelial adhesion of inflammatory cells, reducing the consequence of the increase of endothelial permeability. In fact, a damage of the glycocalyx leads to the passage of larger molecules form the IVS into the ISS, reducing the IVS–ISS oncotic gradient and increasing the ISS fluid volume with tissue edema. Various conditions associated to cardiac surgery may potentially destroy glycocalyx: hemodilution, ischemia and reperfusion damage, and inflammation (Fanzca 2012).
Hemodilution may cause the dissolution of bound plasma protein into the flowing blood and the loss of glycocalyx (Pries et al. 1998; Rehm et al. 2001). The effect may be due to ANP increased levels in cardiac surgery and to fluid overload (Fanzca 2012; Bruegger et al. 2005).
In patients undergoing cardiopulmonary bypass, plasma level of glycocalyx component (heparin sulfate and syndecam-1) was significantly increased with respect to preoperative levels, demonstrating a dangerous effect of ischemia on glycocalyx (Fanzca 2012; Rehm et al. 2007).
In a coronary artery animal model, the loss of glycocalyx due to ischemia leads to an increased permeability to water, albumin, and hydroxyethyl starches (Rehm et al. 2004).
13.2.2 Electrolyte Modifications
Strictly related to fluid management is the management of electrolyte and acid–base balance. Electrolyte alterations are frequent in the postoperative period after cardiac surgery. Polderman and Girbes (2004) compared sodium, potassium, calcium, magnesium, and phosphate levels at the admission to ICU of patient undergone CABG vs. patients undergone other major surgeries. Although all patients received electrolyte intraoperative supplementation, they found a higher percentage (88 % vs. 20 %) of patients with deficit of one or more electrolytes in the first group rather than in the second. These alterations may be due to increased kidney elimination, especially in the case of furosemide and mannitol use, and intracellular shift caused by alteration of ISS, IVS, and ICS equilibrium caused by CPB, extracorporeal circulation, and stress response (SIRS) (Fanzca 2012).
13.3 Clinical Management of Fluid-Therapy
The ideal approach to perioperative fluid management is still debated in cardiac surgery patients. In clinical practice, it may be very difficult: practitioners have to take into account surgical-related factors, such as kind of procedure and duration, CPB duration, SIRS, patient-related factors, such as cardiac disease, presence of reduced ventricular function and its postoperative modification, need for pharmacologic or mechanical assistance, comorbidities (renal and pulmonary dysfunctions are frequent among cardiac surgery patients), and possible complications.
The clinical and literary debate involves different specialists (anesthesiologists, intensivists, and surgeons) and concerns the establishment of the ideal fluid management in terms of kind and amount of fluid to administer.
13.3.1 Which Kind of Fluid?
The choice of the fluid to administer should be guided by the properties of the targeted body space (IVS, ISS, whole body water) (Agrò and Benedetto 2013).
Different solutions are available: crystalloids, colloids, and other fluids, such as dextrose solutions, mannitol solutions, and other concentrated solutions. Each one has its specific indications and side effects (Nuevo et al. 2013).
Historically, the debate about fluid management was developed around the dispute of crystalloids vs colloids. In its course, the medical literature has largely demonstrated the difference in the pharmacokinetics and pharmacodynamics of these two classes of plasma substitutes. Consequently, crystalloids should no longer be considered as an alternative to colloids, and vice versa. Instead, crystalloids and colloids must be considered as two faces of the same coin and their use as part of an integrative fluid management (Agrò and Vennari 2013b).
Recently, the historical debate of crystalloid vs colloid has enlarged to include colloid/colloid discussion. Nonetheless, the physical and chemical properties of various colloids determine the different therapeutic and adverse effects. Thus, any debate about intravenous volume replacement with colloids should consider the potential side effects, involving endothelial integrity, coagulation, platelet function, and organ function (e.g., the kidneys), and not only the effect of the chosen fluid on hemodynamics (Agrò et al. 2013a).
The electrolyte composition of the fluid (crystalloid or colloid) is another source of controversy. The debate on balanced, plasma-adapted solutions started in the 1970s, when their features were first described. A new definition was proposed in 2000 in “Avoiding Iatrogenic Hyperchloremic Acidosis: Call for a New Crystalloid Fluid,” published in Anesthesiology, which referred to the classic need of “a solution containing sodium bicarbonate” because it was clear that “…the predominate physiologic deficit is metabolic acidosis…” (Dorje et al. 2000). Subsequent developments were summarized in 2003 by Reid et al. (2003), who highlighted that scientists and clinicians must inevitably reach a compromise in their long-standing attempts to find the ideal physiological solution.
13.3.2 Physical and Chemical Properties
Depending on their physical and chemical properties, IV solutions may be classified as balanced or unbalanced; plasma adapted or non-plasma adapted; and isotonic, hypertonic, or hypotonic (Agrò and Benedetto 2013).
A balanced, plasma-adapted solution has a composition closer to plasma. It contains sodium, chloride, potassium, magnesium, and calcium in similar concentrations than plasma and contains metabolizable anions, and it is isotonic respect to plasma (same osmolarity). Except for the risk of fluid overload, the infusion of this solution reduces the incidence of side effects related to fluid management, such as metabolic acidosis, electrolytic imbalances, and cellular tone alterations (Agrò and Benedetto 2013).
The infusion of hypotonic or hypertonic solutions will change plasma osmolarity, resulting in a modification of body water distribution. In particular, a hypotonic solution reduces plasma osmotic pressure, since water will move from the ECS to the ICS (Agrò and Benedetto 2013; Williams et al. 1999). Cellular edema and lysis (i.e., hemolysis) may occur (Fig. 13.8). Larger volumes of hypotonic solutions have been known to produce a transient increase in intracranial pressure (ICP), due to cerebral edema (Agrò and Benedetto 2013; Tommasino et al. 1988). The magnitude of this increase can be predicted by the reduction of plasma osmolality (Agrò and Benedetto 2013; Schell et al. 1996). Patients with an osmolality below 240 mOsmol/kg will fall into a coma, with a mortality rate of 50 % (Agrò and Benedetto 2013; Arieff et al. 1976). Consequently, the infusion of large amount of hypotonic solutions should be avoided, except specific cases (Agrò and Benedetto 2013).


Fig. 13.8
Cellular and cerebral edema caused by hypotonic solutions (Agrò and Benedetto 2013, pp. 27–36)
On the other side, hypertonic solutions increase plasma osmotic pressure, moving water from the ICS to the ECS, causing cellular dehydration and, potentially, apoptosis (Agrò and Benedetto 2013) (Fig. 13.9). Many clinical settings may increase plasma osmotic pressure, with a very high mortality (Agrò and Benedetto 2013). Hypovolemic shock triggers hyperglycemia with hyperosmolarity, through the release of epinephrine or through an increase in lactate blood levels (Agrò and Benedetto 2013; Boyd and Mansberger 1968; Järhult 1973; Kenney et al. 1983). It has been shown that in ICU patients, non-survivors had a higher plasma osmolarity than survivors (Agrò and Benedetto 2013; Holtfreter et al. 2006). Moreover, hypertonicity has been shown to make solutions more acidifying. The administration of hypertonic solution reduces strong ion difference (SID) through dilution caused by water movement from ICS to ECS (Makoff et al. 1970) (see Chap. 15).


Fig. 13.9
Hypertonic solutions cause cellular dehydration, leading to apoptosis (From Agrò and Benedetto 2013, pp. 27–36)
13.4 Crystalloids
Crystalloids are low molecular weight salts, dissolved in water. After the infusion, water and salt pass across the body compartment according to physiology (Nuevo et al. 2013).
13.4.1 Classification
Based on their tonicity, crystalloids are classified as hypotonic, isotonic, or hypertonic. There are different generations of crystalloids on the market (Nuevo et al. 2013) (Fig. 13.10). Many studies have focused on producing more balanced and plasma-adapted crystalloids in order to reduce side effect of their use. So far, the rational use of crystalloids remains a clinical problem (Nuevo et al. 2013).
13.4.2 Pharmacokinetics: Distribution and Duration of Action
An isotonic crystalloid is distributed in the IVS (20 %) but mostly in the ISS (80 %). Accordingly, the efficiency of these solutions to expand the plasma volume is only 20 %; the remainder is sequestered in the ISS (Agrò and Benedetto 2013; Reid et al. 2003; Lamke and Liljedahl 1976; Grathwohl et al. 1996; Greenfield et al. 1989; Hauser et al. 1980; Hahn et al. 1997; Takil et al. 2002) (Fig. 13.11). Olsson et al. (2004) found that approximately 30 % of infused crystalloids remain within the IVS for only 30 min. Crystalloids have a short-term volume effect due to their rapid movement from the IVS into the ISS (Nuevo et al. 2013). Thus, the use of crystalloids to replace severe volume deficits, following massive blood or rapid fluid loss, is not effective to restore fluid balance and blood pressure (Drummond and Petrovitch 2005; McIlroy and Kharasch 2003). Attaining the targeted goal of adequate blood pressure requires massive, repetitive infusions, causing the dilution of osmotically active component of plasma and a reduction in plasma oncotic pressure causing further interstitial edema and electrolyte imbalances (Fanzca 2012).


Fig. 13.11
Representation of isotonic crystalloid distribution. IVS intravascular space, ISS interstitial space, ECS extracellular space (Modified from Nuevo et al. 2013, pp. 37–46)
13.4.3 Properties of the Main Crystalloids
The main properties of the most widely used crystalloids are described in Table 13.2 (Nuevo et al. 2013).
Table 13.2
Main properties of crystalloids
Electrolyte or parameter | Plasma | 0,9 % NaCl | Ringer lactate | Ringer acetate | Sterofundin® |
---|---|---|---|---|---|
Colloid osmotic press. (mmHg) | 25 | – | – | – | – |
Osmolality (mOsm/kg) | 287 | 308 | 277 | 256 | 291 |
Sodium (mEq/L) | 142 | 154 | 131 | 130 | 145 |
Potassium (mEq/L) | 4.5 | – | 5,4 | 5 | 4 |
Magnesium (mEq/L) | 1.25 | – | – | 1 | 1 |
Chloride (mEq/L) | 103 | 154 | 112 | 112 | 127 |
Calcium (mEq/L) | 2.5 | – | 1.8 | 1 | 2.5 |
Bicarbonate (mEq/L) | 24 | – | – | – | – |
Lactate (mEq/L) | 1 | – | 28 | – | – |
Acetate/malate(mEq/L) | – | – | – | 27/– | 24–5 |
SID (mEq/L) | 38–42 | 0 | 26 | 26 | 29 |
13.4.4 Normal Saline Solution
The standard 0.9 % NaCl solution is also known as a normal or physiological saline solution. It contains only sodium and chloride, at the same high concentration (Na+ = Cl− = 154 mmol/L) (Nuevo et al. 2013). Consequently, its administration may cause metabolic acidosis and sodium overload. Sodium overload promotes interstitial edema, particularly in the face of the endocrine response to cardiac surgery, the cortisol- and aldosterone-induced sodium retention (Fanzca 2012). This mechanism may be further worsening in patients with kidney dysfunction or postoperative cardiac impairment (Nuevo et al. 2013). Finally, normal saline solution is slightly hypertonic (osm 308 mOsm/kg), potentially providing the tipical complications of hypertonic solutions including a high acidifying power (Nuevo et al. 2013). As a result, normal saline solution is not actually normal, because it is neither isotonic, nor balanced, nor plasma adapted (Nuevo et al. 2013).
13.4.5 Ringer Lactate and Ringer Acetate
Ringer solutions are second-generation crystalloids. Compared to saline solutions, they have less sodium (130 mmol/L) and less chloride (112 mmol/L). They also contain potassium, calcium, magnesium (Ringer acetate), and metabolizable ions: lactate (Ringer lactate) and acetate (Ringer acetate) (Nuevo et al. 2013).
Ringer lactate may interfere with lactate monitoring and may precipitate or aggravate a lactic acidosis, especially in critical cardiac patients (see Chap. 15). Therefore, Ringer acetate is generally preferred (Nuevo et al. 2013). Both Ringer solutions are more plasma adapted than normal saline, but are nonetheless unbalanced (Nuevo et al. 2013).
13.4.6 Latest-Generation Crystalloids
The ionic composition of the latest-generation crystalloids is very close to plasma. They have a lower chloride content than normal saline and have metabolizable anions (acetate, malate). For this reason they differ from Ringer solutions that contain only acetate or malate as metabolizable anions (Nuevo et al. 2013). As a result, these are isotonic, balanced, and plasma-adapted solutions that reduce the risk of cellular osmotic damage (particularly cerebral), of chloride and sodium excess, and dilution acidosis, with a decreased influence on lactate monitoring, lactic acidosis, and base excess (BE) (Nuevo et al. 2013, see Chap. 15).
13.4.7 Potential Risks and Side Effects
13.4.7.1 Water Distribution Modification
Over the past decades, clinicians have routinely restored fluid in the IVS with crystalloids. However, due to the rapid interstitial fluid shifts and the great distribution volume of crystalloids, large doses must be administered. This in turn causes fluid overload, particularly interstitial edema and potential pulmonary edema (they may be deleterious for cardiac surgery patients), as well as metabolic acidosis (Nuevo et al. 2013). Finally, a crystalloid volume-replacement strategy may alter the plasma albumin concentration. Large-volume infusions of crystalloids can lead to albumin hemodilution effects and cause a reduction in colloid oncotic pressure (COP), yet reduced by extracorporeal circulation diluting effects (Fanzca 2012; Nuevo et al. 2013). The reduction of COP alters Starling forces: fluids move from the IVS to ISS and remain in the ISS. The result is interstitial edema up to “compartmental syndrome,” and thus albumin leakage (a vicious circle is established!) with a little improvement of hemodynamics (IVS fluid has not been restored because the infused volume shifts in ISS) (Nuevo et al. 2013; Cervera and Moss 1974). In critically ill patients, a reduction in COP is associated with a mortality rate of approximately 50 % (Nuevo et al. 2013; Morissette et al. 1975; Rackow et al. 1977).
13.4.7.2 Electrolyte Modifications
A large amount of crystalloids infusion may cause hyperchloremia (especially using older generation crystalloid) that alters kidney perfusion leading to sodium and chloride retention. Chloride retention brings to hyperchloremic acidosis further invalidating glomerular filtration rate, while sodium overload causes water retention (Nuevo et al. 2013). The result is a fluid overload that may precipitate the hemodynamic status of the patient, especially in the first hours after the surgery, with a weight gain that may increase mortality (Nuevo et al. 2013; Zander 2009).
13.4.7.3 Clotting Disorders
It is common knowledge that crystalloid administration is an economical means of volume replacement, with an apparently lower risk of clotting disorders (Nuevo et al. 2013). However, in two studies Ruttmann et al. (2001, 2002) and Ng et al. (2002) have shown that in vivo dilution with crystalloids resulted in a significant potentiation of coagulation, due to a decreased concentration of antithrombin III. The resultant hypercoagulability is unrelated to the type of crystalloid. It is also associated with an increased risk of perioperative deep vein thrombosis (Nuevo et al. 2013).
13.4.8 Hypertonic Crystalloids
Hypertonic crystalloid solutions (HCS) contain higher sodium concentrations, ranging from 3 to 7.5 % (Nuevo et al. 2013). According to their high osmolarity, they have a greater expanding volume effect with respect to isotonic crystalloid. HCS may improve the cardiovascular system (especially after CPB-related changes in body fluid compartments due to a capillary permeability) with only a smaller infused volume than isotonic crystalloids (4 mL/kg). However, HCS have a transient volume effect (Nuevo et al. 2013).
Jarvela et al. (2001) studied patient fluid management after CABG surgery. They found that 30 min after the infusion 7.5 % hypertonic saline determined a higher volume expansion with respect to HES 6 %/120/0.7, but lower after 70 and 110 min. HES volume expansion persists for all the observation time, while 7.5 % hypertonic saline volume expansion was shorter. EVS expansion was greater and faster with HCS than with 0,9 % saline solution.
The hemodynamic effects of HCS can be due to the following mechanisms (Nuevo et al. 2013):
Direct myocardial, positive inotropic effect
Direct vasodilator effect (both the systemic and the pulmonary circulation)
Reduced venous capacitance
Fluid shift into the IVS from ISS
Initially, there was enthusiasm in the use of HCS for patients in refractory hypovolemic shock states; At the beginning there was great enthusiasm in the use of HCS, especially in refractory hypovolemic shock states. However they may have life-threatening side effects (Nuevo et al. 2013).
After their infusion, there is a rapid shift of water from EVS into the IVS, without a reduction in COP (McIlroy and Kharasch 2003). An adequate volume status of both the ICS and ECS spaces is necessary to obtain this effect; so that, the prolonged usage of HCS crystalloid is not recommended (Maningas and Bellamy 1986; McIlroy and Kharasch 2003). Both Wade et al. (1997) and Bunn et al. (2002) reported, in their meta-analyses, no significant improvement in outcome in critical patients by using HCS. They hypothesized that the combined used of HCS and colloids would be superior to isotonic fluid resuscitation (Agrò and Vennari 2013b). HCS infusion may be indicated in case of hyposmolarity with hyponatremia (see subsequent paragraphs).
13.5 Colloids
Colloids are high molecular weight molecules that do not dissolve completely in water, nor do they pass freely through the capillary membrane. According to their molecular size, structure, and vessel permeability, colloids determine the oncotic pressure (Agrò et al. 2013b).
13.5.1 Classification
Many colloids have been studied. They include natural colloids (human albumin, HA) and synthetic colloids (dextrans, gelatins, hydroxyethyl starches), differing in their physicochemical properties, pharmacokinetics, clinical effects, and safety. Colloids also can be classified according to their electrolyte composition and tonicity. Consequently, there are balanced and unbalanced colloids, plasma-adapted and non-plasma-adapted colloids, and isotonic and hypertonic colloids (Agrò et al. 2013b).
13.5.2 Physiological Properties of the Main Colloids
The table below describes the main properties of some colloids used in clinical practice (Agrò et al. 2013b) (Table 13.3).
Table 13.3
Properties of some colloid
Electrolyte or parameter | Plasma | Venofundin® 6 % | Gelofusine® 4 % | Tetraspan® 6 % |
---|---|---|---|---|
Colloid osmotic press. (mmHg) | 25 | 37.8 | 33.3 | 37.8 |
Osmolality (mOsm/Kg) | 287 | 308 | 274 | 296 |
Colloid molecule | Albumin | HES 130/0.42 | MFGel | HES 130/0.42 |
Sodium (mEq/L) | 142 | 154 | 154 | 140 |
Potassium (mEq/L) | 4.5 | − | − | 4 |
Magnesium (mEq/L) | 1.25 | − | − | 1 |
Calcium (mEq/L) | 2.5 | − | − | 2.5 |
Chloride (mEq/L) | 103 | 154 | 120 | 118 |
Bicarbonate (mEq/L) | 25 | − | − | − |
Acetate/malate (mEq/L) | − | − | − | 24/5 |
13.5.3 Pharmacokinetics: Distribution and Duration of Action
The IVS volume expansion after the infusion of a colloid depends on the oncotic pressure and the molecular weight (MW), and the higher the oncotic pressure and the higher the MW, the greater the initial volume increase in the intravascular space (IVS) after the infusion. The duration of IVS volume expansion is influenced by MW and organ elimination (mainly kidneys). Thus, different colloids have different durations of volume effects (Agrò et al. 2013b; Mitra and Khandelwal 2009).
Isotonic and iso-oncotic colloids have a lower volume-replacing power, in contrast to hypertonic and hyperoncotic colloids. Based on previous evidence (Agrò et al. 2013b; Nadler et al. 1962), McIlroy and Kharasch (2003) found that an isotonic colloid is distributed only within the IVS. The efficiency of this kind of solution to expand plasma volume is thus 100 % with respect to the infused volume (Agrò et al. 2013b) (Fig. 13.12). However, iso-oncotic, isosmotic colloids rapidly leave the vascular tree, through extravasation or metabolism, especially during certain conditions, such as systemic inflammation, sepsis, capillary leak syndrome, and third-space syndrome, reducing the effective expansion of IVS volume (Chappell et al. 2008; Agrò et al. 2013b; Roberts and Bratton 1998).


Fig. 13.12
Isotonic colloid distribution. IVS intravascular space, ECS extracellular space, EVS extravascular space, ICS intracellular space (From Agrò et al. 2013b, pp. 47–70)
13.6 Natural Colloids: Albumin (HA)
For many years, HA was considered the gold standard in hypovolemia treatment. HA is composed of 585 amino acids with a molecular mass of 69,000 Da. It is the main plasma protein (50–60 %), accounting for 80 % of normal oncotic pressure. Furthermore, HA contributes to the formation of a normal anion gap and acid–base balance while being a charged protein (Agrò et al. 2013b).
13.6.1 Composition and Concentration
Current HA solutions consist of 96 % albumin, with the remaining 4 % being globulins. Different concentrations of HA are commercially available: 20 and 25 % HA (hyperoncotic), 5 % HA (iso-oncotic), and 4 % HA (hyponcotic) (Agrò et al. 2013b).
13.6.2 Pharmacokinetics: Distribution, Elimination, and Duration of Action
A 5 % HA solution can be reasonably considered for volume replacement, leading to an 80 % initial volume expansion, whereas HA 25 % leads to a 200–400 % volume increase within 30 min. The volume effect lasts for 16–24 h (Agrò et al. 2013b; Mitra and Khandelwal 2009). The decrease in the plasma HA concentration is firstly due to passage from the IVS to the EVS through the transporter albondin (transcapillary exchange) and secondly to the fractional degradation rate (Agrò et al. 2013b; Dubois and Vincent 2007).
13.6.3 Clinical Use
There is an extensive literature about the use of HA as the treatment for acute hypovolemia, especially in cardiac surgery. In this class of patients, it has been historically considered the best volume-replacement solution. Additional applications are sepsis, systemic inflammatory response syndrome, and capillary leakage syndromes (Agrò et al. 2013b).
Based on the results of the SAFE study Investigators et al. (2011), HA has been mainly used to treat low plasma protein levels, especially in Austrian and German hospitals. The rationale is to prevent fluid extravasation by increasing the IVS COP in patients at high risk of hypoalbuminemia (Agrò et al. 2013b). Low serum albumin (<2 g/dL) was shown to be a marker of poor outcome, with a mortality of approximately 100 % (Agrò et al. 2013b; Fleck et al. 1985; Marik 1993; Margarson and Soni 1998; Rubin et al. 1997).
HA is not exclusively retained in the IVS: rather, 10 % of the administered dose leaves the IVS within 2 h. It is therefore likely to leak into the ISS, potentially aggravating interstitial edema and hypoalbuminemia, without clinical benefits, especially in patients with increased vessel permeability (cardiac surgery, cardiopulmonary bypass stress response, glycocalyx damages) (Agrò et al. 2013b).
A meta-analysis by Russell et al. (2004) showed HA use yields good results with respect to platelet count as well as a positive influence on oncotic pressure and postoperative weight gain in cardiac surgery patients, with respect to crystalloids.
HA rational use is guided by absolute and relative indications. The administration of HA is indicated in acute conditions requiring plasma expansion and in chronic conditions characterized by low albumin plasma levels (Vincent et al. 2003). There is widespread consensus in the literature and in clinic regarding absolute indications. Relative indications refer to settings in which HA is indicated when other specific criteria are satisfied (Agrò et al. 2013b).
The cardiac surgery is a relative indication to HA use. Particularly in hypoalbuminemic cardiac surgery patients, even if the clinical benefits are not yet clear (Agrò et al. 2013b). Another relative indication that may be present in cardiac surgery patients is hemorrhagic shock (Agrò et al. 2013b).
Albumin is routinely administered, albeit improperly, in post-cardiac surgery ICU patients in case of (Agrò et al. 2013b):
Hypoalbuminemia in the absence of peripheral edema or acute hypotension
Malnutrition and malabsorption
Wound healing
Nonhemorrhagic shock
Diuretic-responsive ascites
Hemodialysis
Ischemic stroke.
HA use in the cardiac surgery patient is expensive and the clinical advantages achievable do not seem to justify its cost (Agrò et al. 2013b).
13.6.4 Potential Risks and Side Effects
Several lines of evidence explain why HA supplementation may worsen the ICU patient condition (Agrò et al. 2013b). In fact, after rapid volume replacement, cardiac failure may occur, causing or worsening pulmonary edema, especially in capillary leak syndrome (Agrò et al. 2013b; Kaminski and Williams 1990). Furthermore, HA may impact coagulation and hemostasis by enhancing antithrombin III activity and inhibiting platelet function (Agrò et al. 2013b; Rajnish et al. 2004). Tobias et al. (1998) found that albumin may also lead to hypocoagulability. Dietrich et al. (1990) showed an in vitro increase in bleeding time, which may increase blood loss in postsurgical patients. Finally, albumin administration may impair the efficiency of endothelial cell adhesion. The importance of this effect is uncertain, since increased plasma levels of endothelial adhesion molecules may be markers of mortality (Agrò et al. 2013b; Kaminski and Williams 1990).
In acute renal failure, HA may accumulate after its massive administration (Agrò et al. 2013b; Kaminski and Williams 1990).
HA is generally well tolerated, but immediate allergic reactions are possible, consisting in fever, nausea, vomiting, pruritus, hypotension, and even cardiorespiratory collapse (Agrò et al. 2013b).
13.7 Synthetic Colloids
Synthetic or artificial colloids (dextrans, gelatins, hydroxyethyl starches) are produced from biological, nonhuman molecules. Their assessment criteria include (Agrò et al. 2013b) (Table 13.4):
Table 13.4
Comparison between therapeutic and side effects of artificial colloids
Colloid | Volemic effect | Side effect | |||
---|---|---|---|---|---|
Efficacy | Duration | AKI | Coag. | Anaph. | |
Dextrans | +++ | +++ | +++(40) | +++(70) | ++ |
Gelatins | + | + | + | + | +++ |
HES high MMW | +++ | +++ | ++ | +++ | + |
HES low MMW | +++ | ++ | + | ++ | + |
Concentration
Initial volume effect
Duration of the volume effect
Side effects
13.8 Dextrans
Dextrans are glucose polymers of different sizes, derived from Leuconostoc mesenteroides, a bacterium originally isolated from contaminated sugar beets (Agrò et al. 2013b).
Dextrans are mainly used in the USA, as they are no longer available in European countries. The most widely used dextran solutions are dextran 40 (a 10 % solution with 40,000 mean MW) and dextran 70 (a 6 % solution with 70,000 mean MW) (Agrò et al. 2013b) (Table 13.5).
Table 13.5
Main properties of dextrans
Characteristics of dextran solutions | 6 % Dextran 70 | 10 % Dextran 40 |
---|---|---|
Mean molecular weight (Dalton) | 70,000 | 40,000 |
Volume efficacy (%) | 100 | 175–(200) |
Volume effect (hours) | 5 | 3–4 |
Maximum daily dose (g/kg) | 1.5 | 1.5 |
13.8.1 Pharmacokinetics: Distribution, Elimination, and Duration of Action
Dextrans are endowed with a high COP, due to their high water-binding capacity. After the infusion, dextrans lead to a 100–150 % increase in the volume of the IVS (Agrò et al. 2013b; Mitra and Khandelwal 2009). They are mainly eliminated by the kidney, while only a small fraction transiently passes into the ISS or is eliminated by the gastrointestinal tract. In particular, smaller molecules (14,000–18,000 kDa) are excreted by the kidneys within 15 min, whereas larger molecules are excreted after several days. At 12 h from administration, 60 % of dextran 40 and 30 % of dextran 70 has already been eliminated (Agrò et al. 2013b; Mitra and Khandelwal 2009; Arthurson et al. 1964; Atik 1967).
13.8.2 Clinical Use
Dextran solutions may be suitable in post-cardiac surgery ICU patients because they have positive effects on circulation. In fact, they have been shown to adequately restore and maintain hemodynamic in case of shock and to ameliorate tissue perfusion and microcirculation. At the same degree of hemodilution, rheological effects are mainly correlated with the use dextran 40 rather than with any other plasma substitute. Moreover, dextran protects against ischemia–reperfusion injury by reducing the harmful interactions between activated leukocytes and the microvascular endothelium (Agrò et al. 2013b).
13.8.3 Potential Risks and Side Effects
Despite evidence on improvement of macro- and microcirculation after infusion, dextrans are no longer used because of their side effects (Agrò et al. 2013b).
Dextrans administration may lead to anaphylactoid reactions more frequently and more severely than other colloids. This is due to the massive production of vasoactive mediators triggered by anti-dextran antibodies. These reactions may be prevented by pretreatment of the solution with 20 mL of hapten (dextran 1,000) few minutes before infusion (Agrò et al. 2013b; Allhoff and Lenhart 1993).
Another possible side effect is renal dysfunction and AKI, through the production of hyperviscous urines leading to swelling and vacuolization of tubular cells and tubular plugging. This is especially true in patients with advanced age, hemodynamic alterations, preexisting renal disease, and dehydration (Agrò et al. 2013b; Mitra and Khandelwal 2009; Baron 2000; Moran and Kapsner 1987).
Finally, dextrans may alter platelet function, decrease factor VIII levels, and increase fibrinolysis, with significant bleeding disorders, especially after the administration of high doses (Agrò et al. 2013b; Mitra and Khandelwal 2009; Barron et al. 2004). These side effects resulted in maximum daily dose recommendation of approximately 1.5 L (Feng et al. 2006).
Currently, dextrans have a very limited use in the clinical practice, especially in ICU patients who present many risk factors for the development of dextran side effects (Agrò et al. 2013b).
13.9 Gelatins
Gelatins are polydispersed peptides derived from bovine collagen. Three types of gelatins are currently available: cross-linked or oxypolygelatins (e.g., Gelofundiol), urea-cross-linked gelatins (e.g., Haemagel), and succinylated or modified fluid gelatins (e.g., Gelofusine) (Table 13.6). Their average MW is 30–35,000 Da, and they are based on unbalanced, hypotonic solutions. In particular, polygelines are dispersed in a 3.5 % polyelectrolyte solution generally containing Na+ 145 mEq/L, K+ 5.1 mEq/L, Ca2+ 6.25 mEq/L, and Cl− 145 mEq/L. Thus, they may increase serum calcium, in particular after large-volume infusions. Succinylated gelatins are dispersed in a 4 % polyelectrolyte solution generally containing Na+ 154 mEq/L, K+ 0.4 mEq/L, Ca2+ 0.4 mEq/L, and Cl−120 mEq/L (effective SID = 34). Their low chloride content reduces the risk of hyperchloremic acidosis and may be helpful in patients with acid–base alterations (see Chap. 15). They are compatible with blood transfusions because of their low calcium content (Agrò et al. 2013b; Mitra and Khandelwal 2009).
Table 13.6
Main properties of gelatins
Characteristics of gelatin solutions | Succinylated gelatins | Cross-linked gelatins | Urea-cross-linked gelatins |
---|---|---|---|
Concentration (%) | 4.0 | 5.5 | 3.5 |
Mean molecular weight (Dalton) | 30,000 | 30,000 | 35,000 |
Volume efficacy (%) | 80 | 80 | 80 |
Volume effect in hours | 1–3 | 1–3 | 1–3 |
Osmolarity (mOsm/L) | 274 | 296 | 301 |
13.9.1 Pharmacokinetics: Distribution, Elimination, and Duration of Effect
Gelatins have similar IVS volume-expanding power and a half-life of about 2.5 h. After 24 h post-administration, 13 % remains in the IVS, 16 % has passed into the ISS, 71 % is rapidly cleared by the kidneys, and a small amount has been cleaved by proteases in the reticuloendothelial system (RES). Notably, the volume expansion is lower than the infused volume (about 70–80 %). Gelatins have the shortest duration of effect than any other colloids. Therefore, repeated infusions are required and allowed by their rapid elimination, as there are no dose limitations, in contrast to other colloids (Agrò et al. 2013b; Mitra and Khandelwal 2009; Barron et al. 2004).
13.9.2 Potential Risks and Side Effects
In ICU patients and in patients with severe hemorrhagic shock, who need large intravascular volume replacement, gelatin solutions are still widely adopted because of the lack of accumulation in the reticuloendothelial system (RES), the unlimited dose, and the absence of significant side effects on kidney function. Conversely, they are the second most frequent cause of anaphylactic shock in cardiac surgery patients, following antibiotics (Agrò et al. 2013b; Barron et al. 2004).
Historically, gelatins have been considered safer than other colloid with respect to bleeding. However, recently, there has been evidence of platelet dysfunction and clotting disorders (Agrò et al. 2013b). In a study comparing the effects of progressive hemodilution with gelatins, saline, hydroxyethyl starches, and albumin on blood coagulation, significant changes in the thromboelastogram were found after the infusion of gelatin solutions (Adamson 2008). Nonetheless, in clinical practice, they seem to impair fibrin polymerization less than the “modern” medium molecular weight starches (Agrò et al. 2013b).
13.10 Hydroxyethyl Starches
Hydroxyethyl starches (HES) are modified natural polysaccharides derived from amylopectin, a highly branched starch similar to glycogen, derived from maize or potatoes. Polymerized d-glucose units are connected by 1–4 linkages with one 1–6 branching linkage every 20 glucose units. Natural starches cannot be used in clinical routine since they are rapidly hydrolyzed by circulating α-amylases (Fig. 13.13). HES are obtained by replacing the hydroxyl groups of natural starches with hydroxyethyl groups at the C2, C3, and C6 carbon positions of anhydroglucose residues. A greater solubility and less amylase degradation are obtained, especially for hydroxyethyl groups at the C2 position (Agrò et al. 2013b; Mitra and Khandelwal 2009).
13.10.1 Classification
The first HES was produced in the 1970s in the USA. Since then, further generations have been produced. HES are designated by a series of numeric parameters (e.g., HES 10 % 200/0.5/5) reflecting their pharmacokinetics. The first number relates to the solution concentration, the second represents the mean MW (MMW), the third is the molar substitution rate (MSR), and the fourth is the C2:C6 ratio. Thus, HES may be classified according to (Agrò et al. 2013b):
Concentration (3 %, 6 %, 10 %)
MMW (low molecular weight, 70 kDa; medium molecular weight, 130–270 kDa; high molecular weight, >450 kDa)
MSR (low MS, 0.4–0.5; high MS, 0.62–0.7)
C2:C6 ratio
The volume-expansion power of HES is firstly influenced by concentration. HES at 6 % concentration are iso-oncotic and have a 100 % volume-expanding power (1 L of fluid infused = 1 L of plasma volume expansion). HES at 10 % concentration are hyperoncotic and have a volume-expanding power >100 % (1 L of fluid infused = >1 L of plasma volume expansion) (Agrò et al. 2013b; Mitra and Khandelwal 2009; Dubois and Vincent 2007).
HES are polydispersed solutions made up of different-sized molecules. Particles with a low MW (45–70 kDa) have a rapid enzymatic degradation and a fast renal excretion, because their size is under the renal threshold. Particles with high MW (>70 kDa) have a longer half-life, according to both their size and their rate of enzymatic degradation. The combined effect of the particles with different metabolism influences duration of volemic effect after HES infusion (Agrò et al. 2013b; Barron et al. 2004).
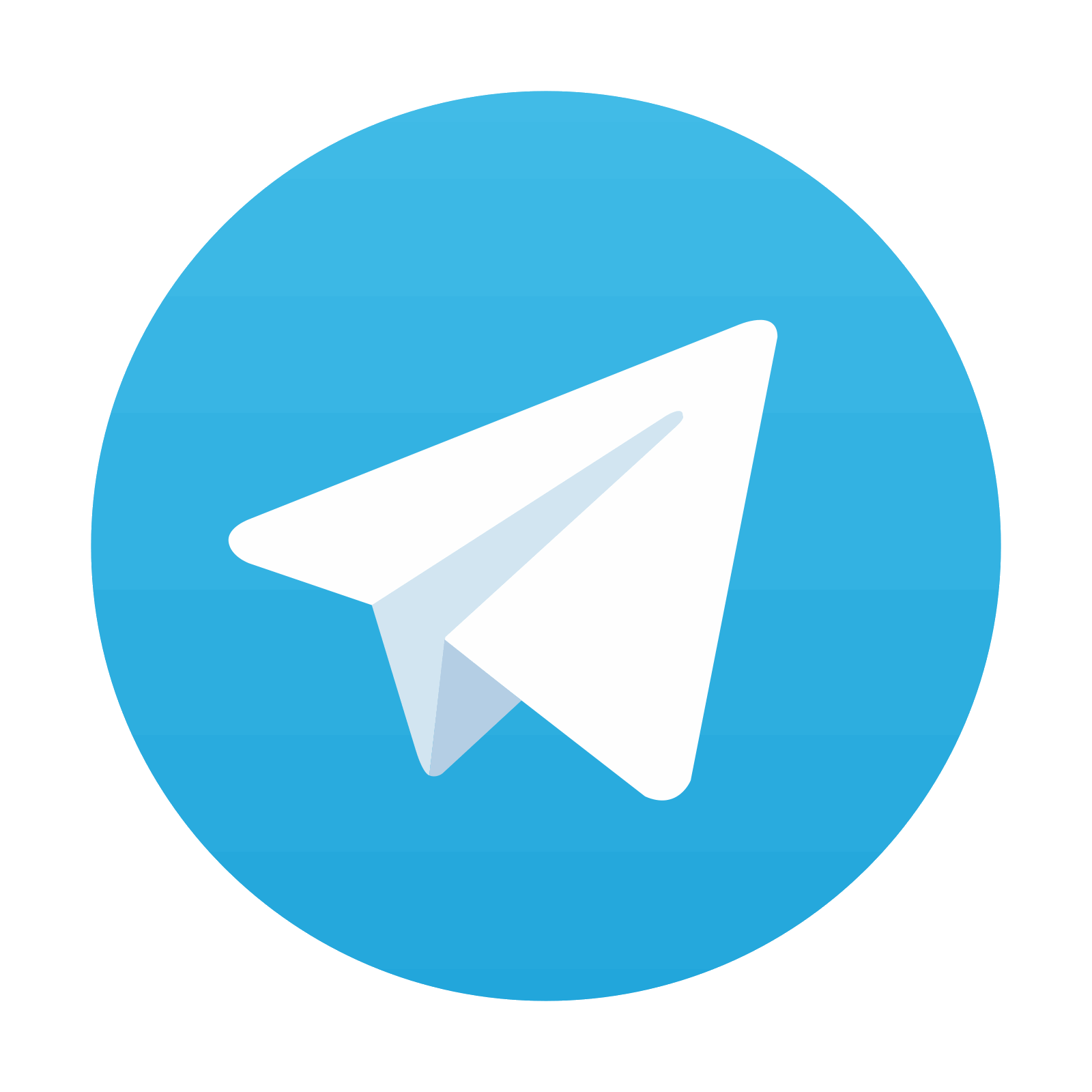
Stay updated, free articles. Join our Telegram channel
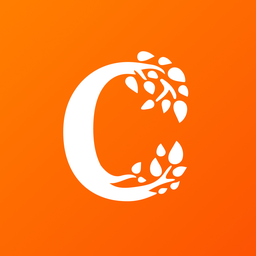
Full access? Get Clinical Tree
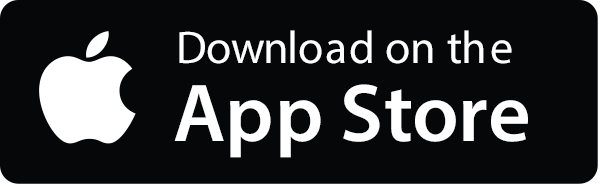
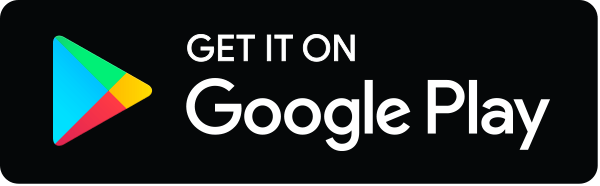